Laser based hard X-ray generation
Short-pulse high-power laser systems make it possible to produce hot plasmas of solid-state density. Early experiments using sub-nanosecond pulses demonstrated the generation of X-ray radiation from these plasmas. Today, chirped amplified Ti:sapphire lasers achieve pulse energies of more than 250 mJ and durations of 100 fs or less in table top set-ups. At relativistic intensities (I > 1018 W/cm2), hard X-rays with photon energies exceeding 1 MeV are produced when this radiation interacts with a solid or liquid target. In contrast, small-scale, short-pulse hard X-ray radiation sources of characteristic X-ray line radiation in the 2-25 keV region promise to be a powerful new tool in physics as well as in chemistry, biology and material science.
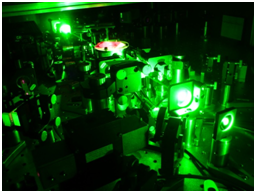
Available laser system
- mirror dispersion controlled, soft aperture kerr lens mode locked Ti:sapphire oscillator, 80 MHz, > 3 nJ, sub 12 fs
- 9-pass chirped pulse amplifier, 1 kHz, > 500 µJ, sub 28 fs
- 4-pass cryogenic CPA-system, 1 kHz, > 5 mJ, sub 30 fs, > 100 GW
In the weakly relativistic regime (I < 1018 W/cm2), spectral analysis of the generated radiation shows features similar to those known from conventional X-ray tubes, namely a polychromatic continuum and characteristic line radiation. This latter component is of interest for applications due to its well-defined emission wavelength, the narrow spectral line width, the high intensity and its potential high brightness. These similarities are not surprising since in both cases the stopping of high-kinetic-energy electrons leads to radiation emission. However, the situation for laser-produced X-rays is more complicated due to plasma processes and interactions with the intense electric and magnetic fields of light pulses. Furthermore, the radiation is not only generated inside the solid but also in the plasma. The observed emission spectrum is therefore the result of many different processes. In a simple picture, two main processes are responsible for the X-ray emission. The interaction between thermal electrons and ions in the laser-generated plasma leads to a thermal X-ray component. Additionally, super-thermal electrons, termed ‘hot-electrons’, are caused by the interaction of the ultra-high light intensities with the dense plasma. Line radiation of highly charged ions, for example Heα-like radiation, can be produced in the plasma by these high-kinetic-energy electrons. Due to their long mean free path, fast electrons can penetrate into the cold region of the solid target material, producing either Bremsstrahlung via collisions or (cold) line radiation by knocking out bound K-shell electrons of neutral target atoms.
Using this technique ultra-short (< 1 ps) hard X-ray pulses are generated. By changing the target material discrete photon energies can be produced at 1486 eV and 1557 eV (Al Kα,β), 6404 eV and 7058 eV (Fe Kα,β) or 8048 eV and 8905 eV (Cu Kα,β).
Applications
In two projects founded by the BMBF (Bundesministerium für Bildung und Forschung) we investigate the potential of these laser-based hard X-ray sources to contribute to sate of the art analyzing technologies like hard X-ray photoemission spectroscopy (HAXPES) and photoemission electron microscopy (PEEM).
Special unique X-ray optics like toroidally bent single crystals or elliptic multilayer mirrors harvest the generated X-ray light for further applications. Focused down to a sample prepared in a vacuum chamber, the X-ray photons interact with the material via the photo effect and discharge electrons. Spectral analysis results in chemical information about the sample. Imaging of the electrons provides the surface structure. A combination of both techniques e.g. time-of-flight X-ray photoemission electron microscopy (TOF-X-PEEM) benefits from the pulsed character of the excitation X-ray source and its high repetition rate.
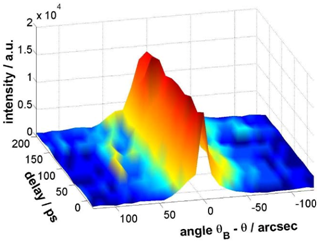
A second application is time-resolved X-ray diffraction (TR-XRD) employing the pump-probe technique. A crystal lattice is excited using a laser pump pulse and the temporal evolution of the lattice vibration is measured by a delayed X-ray probe pulse. The time delay between these pulses is variable and therefore a time resolved measurement of the lattice vibration can be done resulting in a sequence of diffraction patterns. By evaluating this series of diffraction patterns, the duration of the process can be estimated. The temporal resolution in such an experiment depends in general on the pulse duration of both applied pulses, which is excellent (sub 30 fs optical and sub ps X-ray) in our case. Additionally the two pulses are intrinsically synchronized due to their common source.